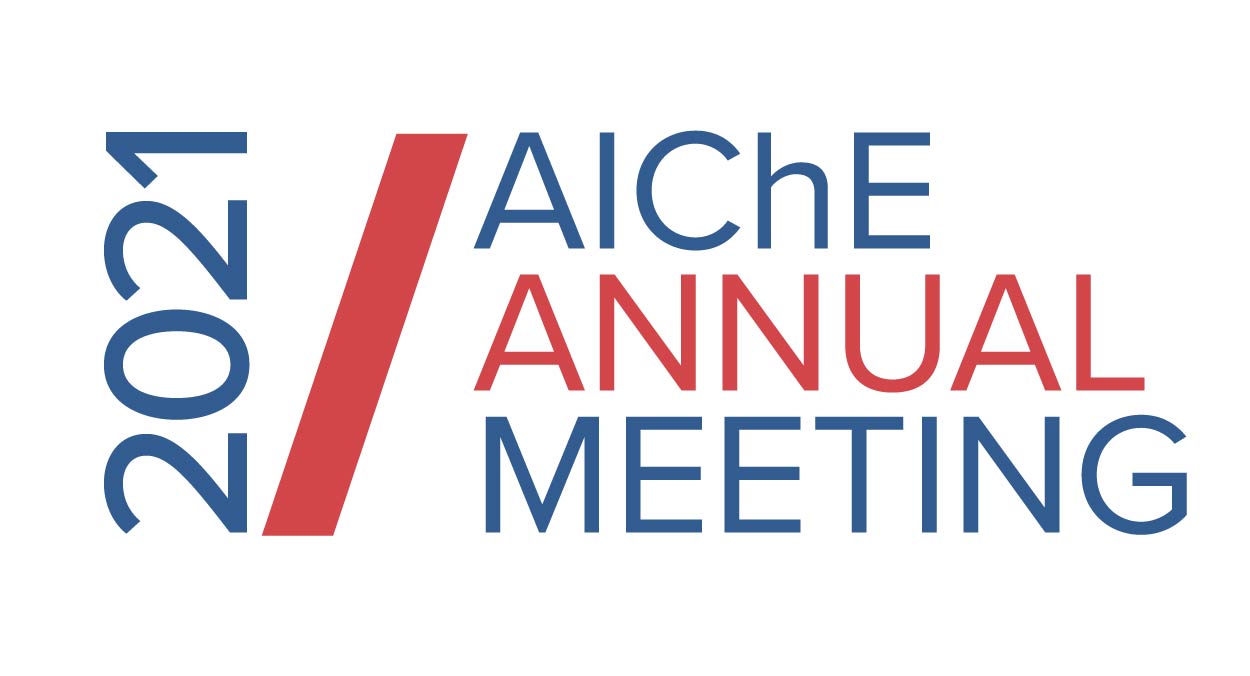
AIChE will undertake a routine upgrade of our digital infrastructure between 12AM to 6AM ET on Tuesday April 22. During this time access to all services that require login or payment will be unavailable.
Here, we introduce a new approach to achieve osteoblast-to-osteocyte differentiation applying biomaterials and tissue-engineering strategies. Osteocytogenesis begins with osteoblasts that actively secrete collagen fibers and synthesize mineralized matrix. Thus, we hypothesize that the progressive changes in the mechanical and physical environment is required to induce osteocyte differentiation pathway in vitro. To test this hypothesis, we fabricated semi-transparent demineralized bone paper (DBP) from bovine bone through the rapid demineralization process that we devised previously (2). Briefly, bovine compact bone block is demineralized in 1.2 N hydrochloric acid (HCl) solution with cyclic pressure change up to 4 bar. This process demineralized the 5 mm bone matrix in 5 days. Then, a demineralized bone block was cryosectioned 20 um in thickness, which is a similar dimension of lamellae of natural bone. We confirmed that DBP, a bone-derived biomaterial, supports intrinsic phenotype and interaction of osteoblasts and osteoclasts in vitro. Especially osteoblasts rapidly deposited structured minerals on DBP, indicating that lamellar structure of dense collagen of DBP served as a functional template for guided mineralization. However, it took around 3 months to find the cells buried in the mineralized matrix when they were cultured on a single layer of DBP. Thus, we stacked 5 layers of DBP to reproduce the embedding osteoblasts, which are the early stage of osteoblast-to-osteocyte transition (Fig. 1a). In the multi-layered DBPs, osteoblasts keep mineralizing the surrounding collagen matrix and eventually entrapped between mineral layers. After 1 month of culture, we found that cell density decreased due to the dramatic change of microenvironments during the embedding process. However, the cells that adapted to the newly established environment showed the transition from an elongated osteoblast to a highly dendritic morphology, which is a unique characteristic of osteocytes (Fig. 1b). Furthermore, dendrites of the cells were elongated and connected to the neighboring cells. Those dendritic cells also showed the up-regulated DMP-1 and Sost expressions that are well-known osteocyte markers. Overall, the embedding process in a layer-by-layer manner could reproduce the transition from osteoblasts to osteocytes in vitro.
We further characterized the effect of hypoxic condition in osteocytogenesis. When bone forms in the body, it is regarded that embedded osteoblasts experience the radical shift of oxygen tension. We stimulated reduced oxygen tension (2 %) by culturing stacks of DBPs with osteoblasts in the hypoxic chamber. There hasnât been much change in cell density and dendritic cellular processes, but mineralization and embedding processes in hypoxic condition occur more quickly than normoxic conditions. As a consequence, the cells within DBP stacks showed higher DMP-1 gene expressions in the same time period of culture. This result indicates that oxygen tension in the bone microenvironment is another factor that enhances osteocytogenesis.
Recent research has shown that adaptive responses to mechanical stimulation in bone play a critical role in the regulation of bone homeostasis and cell activities. There has also been a considerable amount of evidence showing that osteocytes act as mechanosensors to control bone cell functions. Mechanical loading stimulates the mechanosensation and mechanotransduction of osteocytes as well as the mechanical strain signal transmitted to surface of bone cells via the lacunocanalicular system. Hence, we hypothesize that transmitted mechanical stress is required to regulate transitional osteocyte differentiation. We tested this hypothesis by fabricating a bioreactor applying cyclic mechanical compression to multiple layers of osteoblast-seeded DBPs (Fig. 1c). Specifically, we built this bioreactor by integrating acrylic plates with a magnet, a pneumatic piston, solenoid valves, and a programmable controller. With the help of airflow, the pneumatic piston raises the acrylic plate with magnets to the bottom of a 24-well plate. When those magnets approach the bottom of the plate, the magnet inserts (2.5 mm in diameter) in each well are pulled downward via magnetic response. When the airflow is restricted, the magnet plate descends as a result of gravity and the magnetic attraction between the inserts and the plate is released. The cyclic movement of the magnet plate is controlled by a programmable controller connected to a solenoid valve to turn the airflow on or off into the bioreactor. A cyclic compression force can be applied on the DBP stacks by placing them under the magnet inserts in the plate. We tested the effect of cyclic mechanical stress by applying the compression force every minute for 10 seconds. As with previous embedding and hypoxic tests, confocal microscopy images with actin staining showed that the cell density of the internal layers slightly decreased after mechanoculturing under hypoxic conditions. However, the surviving osteoblasts were embedded in a mineralized matrix and converted into dendritic osteocytes (Fig. 1d). Furthermore, the stronger force was applied on DBP stacks by increasing the size of magnet inserts from 2.5mm to 3.9 mm in diameter, the higher Sost gene was expressed after mechanoculturing in hypoxic condition (Fig. 1e). This result indicates the loading intensity could be one of regulators of osteocyte differentiation and their activities. Overall, osteoblast-to-osteocyte differentiation was induced by mimicking mechanical microenvironment of bone tissue in vitro. To our knowledge, this is the first demonstration of osteoblast-to-osteocyte differentiation reproduced by only applying physical factors.
Moreover, we tested human osteocyte differentiation with the established mechanoculturing protocol by replacing bovine bone and mouse osteoblasts to human bone and osteoblast. Likewise, human osteoblasts showed the similar embedding process and their dendritic process, which is consistent with the mouse model. As expected, hypoxic condition and mechanical stress result in the highest Sost expression from human cells in multi-layered DBPs (Fig. 1f). Recent research has not been extended to the human osteocytes and their functional activities. This humanized osteocyte model provides a unique opportunity to investigate human bone biology in multiple dimensions and facilitates preclinical translational research.
Finally, we observed osteocyte-like morphology changes in osteoblasts derived from DsRed transgenic mice and humans within stacks of the semitransparent DBP. Actin filament staining confirmed extensive dendritic cellular processes that are representative of the osteocyte phenotype. Furthermore, DMP-1 and SOST gene expressions of isolated osteoblasts highly increased after mechanoculturing under hypoxic condition, indicating that mechanical stress and hypoxic condition accelerate the osteocyte differentiation by making similar microenvironment to in vivo condition. We envision that the established reproducible and functional osteocyte differentiation protocol will delineate the transitional osteocyte differentiation and their functional regulation of the bone surface cell activity.
References
1. Dallas et al., The Osteocyte: An Endocrine Cell . . . and More, Endocr. Rev. 34 (2013)
2. Park et al., Trabecular bone organoid model for studying the regulation of localized bone remodeling, Sci. Adv. 7:eabd6495 (2021)